Benzene (left) and aspartyl protease inhibitors (right) showing an iso-contour through their respective electron densities.
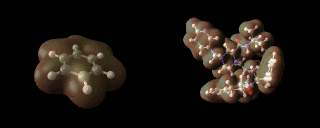 |
Atoms are electrically neutral; the negative charge of
the electrons balances the positive charge of the
nucleus. But almost all atoms bind both to other atoms of
the same type and to different atoms. (The exceptions to
this rule are of particular interest as discussed below.)
The science of chemistry is largely concerned with atomic
aggregates (which when they involve a small number of
atoms are, as we learned earlier, called molecules). It
is at first sight very difficult to understand why these
electrically neutral entities should stick to each other
so strongly and so comprehensively. Indeed, the nature of
the bonding between atoms is the most fundamental problem
in chemistry. It is, however, a problem that has been
solved, and one to which modern computational methods
have made an essential contribution.
The key to understanding chemical bonding is the ability
to explore the complex rearrangements of the
atomic electron clouds which occur when atoms interact.
As we have seen, atoms are themselves very complicated;
and we can only write down an exact mathematical solution
of the Schrödinger equation for the simplest of cases,
the hydrogen atom. For all other atoms we must use
approximate solutions (which can, however, achieve a high
level of accuracy) whose derivation requires intensive
numerical work for which a computer is ideal. Molecules
represent an even higher order of complexity; and
computational methods are essential if we are to solve
the Schrödinger equation at a sufficient level of
accuracy. This can now be achieved and
accurate wave functions are widely
available for molecules containing an increasingly large
number of atoms. The image above, for example,
illustrates the calculated electron densities in the
benzene molecule and a pharmaceutical molecule to which
we return later.
Colour coded contours showing the electron density about two hydrogen atoms (shown in white) in a hydrogen (H2) molecule.
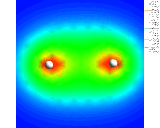 |
The atomic electron clouds rearrange when atoms interact
because, of course, the atoms perturb or influence each
other. The rearrangement occurs almost entirely in the
highest energy orbitals or outermost shell of electrons;
electrons in the lower energy states are too strongly
bound to their nuclei. It is indeed fair to say that
chemistry is about what goes on at the margins of atoms.
There are three broad classes of rearrangement which we
will denote as concentration, transfer and
delocalisation (or spreading out), which are
responsible for the different types of chemical bonding.
Concentration is the most universal form of electron
redistribution. It involves electron density being
drained away from the outermost orbitals of the
interacting atoms and concentrated in the region between
the nuclei. Here the negatively charged electrons are
close to the positive nuclei of both atoms and
therefore have a low energy (because, of course, positive
attracts negative). Indeed, the enhanced electron density
in the region can be thought of as a sort of glue which
holds the atoms together. This type of bonding is
illustrated above for the simplest of all assemblies, the
hydrogen molecule, (H2) - two hydrogen
atoms stuck together. We show a
contour plot of the calculated electron density; The
second shows a more subtly revealing difference contour
plot which represents the difference in the electron
density in the molecule compared with that for two
non-interacting atoms. Enhancement of the density between
the nuclei and depletion around the peripheries of the
atom is clearly seen.
Eletron density build up along the covalent bonds of ethane.
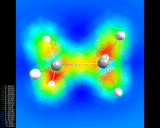 |
A 'difference' density map for two hydrogen atoms in a hydrogen (H2) molecule showing increased electron density between the atoms.
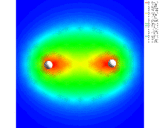 |
Chemists have traditionally assigned the term covalence
to this type of bonding which is very widespread. The
bonds between carbon atoms in the diverse ring and chain
compounds formed by this element - compounds which
are of immense industrial importance and which are found
in our bodies and those of all living matter - are
covalent in nature. Moreover, the silicon atoms in
crystalline silicon - the material at the heart of
the ongoing electronics revolution - are again held
together by covalence as illustrated below.
Covalent bonding - the possibility of
draining off a small amount of electron density from the
edges of atoms and concentrating it as a 'glue' between
them - makes both life and the electronic age
possible.
Covalent bonding in the structure of silicon.
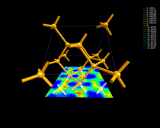 |
The electron density differences within sodium chloride, NaCl.
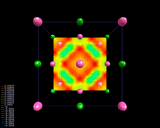 |
Our next mode of density rearrangement - transfer between different
atoms - is even easier to understand. Different atoms have different affinities
for electrons. For example, atoms like oxygen, fluorine and chlorine tend
to pull electrons into their outermost orbitals; while atoms such as sodium,
potassium and magnesium only hold their electrons in these orbitals weakly.
The greatest of all twentieth century chemists, Linus Pauling, coined the
term electronegativity to quantify the electron attracting powers
of atoms; a more detailed discussion is given in the Glossary. Thus low
'electronegativity' atoms like sodium will easily lose or transfer electron
density from its outermost orbital to a high electronegativity chlorine
atom. The sodium atom will then have a positive charge and the chlorine
a negative charge. These oppositely charged atoms, which are given the
name ions, attract each other. This simple process of transfer of
electron density with accompanying electrostatic binding between oppositely
charges ions is known as ionic bonding. It is illustrated
on the left for the case of sodium chloride (NaCl), the archetypal
ionic compound.
As is the case with most ionically bonded systems, NaCl forms a crystal
structure in which there is a regular arrangement of atoms in three
dimensions.
The figure on the left displays
difference contour maps which clearly show the transfer of electron density
from the sodium to the chlorine. A more subtle example is provided by quartz,
an important, naturally occurring mineral and industrial material which
has the chemical formula SiO2. The image shows how density
is redistributed in this compound. Clearly there is transfer of density
from silicon to oxygen; but there are some indications of concentration
of density between the nuclei. Quartz shows bonding that is intermediate
between ionicity and covalence. Both silicon and oxygen are widespread
in the earth's crust and mantle; and together with atoms of metallic elements
they form an enormously diverse range of silicate compounds.
The partially ionic silicon-oxygen
bond is therefore of equal importance to the mineralogist as is the carbon-carbon
bond to organic and biochemists.
The electron density differences within quartz, SiO2.
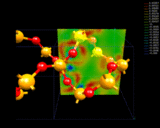 |
Our third type of electron density redistribution is delocalisation;
spreading out of electron density over several atoms results in a reduction
in the electronic energy. The classic example is benzene, the hexagonal
ring structure molecule illustrated on the left (and which was first
isolated from whale oil by Michael Faraday in The Royal Institution in
1825). The molecule shows considerably greater stability - is far less
reactive - than might be expected. Understanding this phenomenon was one
of the major challenges in the development of theoretical organic chemistry.
We now know that this stability is conferred by some of the electron density
in the molecule spreading out over the whole ring as illustrated.
Delocalisation is also the basis of the bonding between metal atoms.
When, for example, sodium atoms come together to form a metallic crystal,
the electrons from the outermost orbitals become delocalised over the entire crystal,
as shown on the left. And it is the existence of the delocalised 'sea'
of electrons which results in the characteristic high electrical conductivity
of the metal. Interestingly, it is possible to produce one dimensional
metals from chains of carbon atoms. These 'polyacetylenes'
have very high metallic-like electrical conductivities,
but with mechanical properties more like those of plastics.
Delocalized molecular orbitals in benzene.
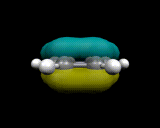 |
A packed assembly of polyacetylene molecules.
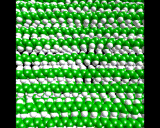 |
Sodium atoms in metallic sodium.
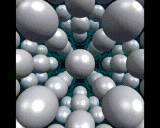 |
All chemical bonding thus involves one or more of those three modes
of redistribution of the loosely bound outermost electrons so as to lower
the energy of the resulting atomic assembly. Moreover, this process is
one which can be modeled in great detail and with high accuracy using
computational methods. The problem of the chemical bond is solved!